Nuclear Physics and Nuclear Energy, Part 2
- WSP Rhodes
- Jun 19, 2022
- 11 min read
If you haven’t read part 1 yet, I would encourage you to do so. This time, I will talk about how nuclear reactors work and give an accurate, nuanced take on the risks that are posed by nuclear energy and by its absence. Before we begin, I feel I should mention that this post is affected by my views on nuclear energy based on known science. Polls show that 79% of physicists and 75% of engineers support the construction of more nuclear power plants, and while this is a super majority, it’s not like the 97% of climatologists who believe in man-made climate change. This is a somewhat contentious issue among climate scientists and activists for reasons I will touch on. So my goal with this post will not be to say that nuclear energy is uncomplicatedly good, but to say that the risks it poses are manageable and its benefits are at least worth considering.
How Reactors Work
My previous post discussed how nuclear chain reactions work in both nuclear weapons and energy. This time, I will focus on how these reactions work in nuclear reactors specifically. In all cases, the heat generated from a nuclear reaction is used to turn water into steam to turn turbines, similar to coal power plants. Of course, there are many ways to design a nuclear reactor, but there are three parts that all reactors need:
Fuel; the part of the reactor that actually produces the chain reactions needed for energy. This is usually uranium that has been enriched by some amount, but can also be plutonium. This fuel is shaped into pellets that are stacked atop each other and encased in rods of zirconium. Each rod is individually below critical mass, but a critical mass can be created by arranging many rods next to each other.
Control rods; rods that sit between the fuel rods and prevent any chain reactions. These rods are made from materials such as cadmium or boron that absorb neutron radiation, essentially isolating the fuel rods from each other so they don’t become a critical mass. The control rods in a reactor are raised or lowered by levers or pulleys to allow or inhibit the chain reaction and control the amount of energy being produced.
Moderator; I very briefly mentioned last time that the speed of the radiation particles hitting a fissile atom impacts how likely it is to trigger that atom to fission. For Uranium-235 and other nuclear fuels, the radiation that comes off fuel atoms is actually going faster than is ideal to set off fission. These fast neutrons can set off fissions, but they’re not as likely to do so as neutrons moving only 1/10,000th of this speed. This isn’t as much of a concern in nuclear weapons as the fuel is so enriched that even a low probability of fissions will create a runaway fission reaction, but the less enriched fuel in a reactor needs more help to reach criticality. Moderators are materials that slow neutrons down without absorbing them, thus increasing the rate of nuclear reactions. (If the idea that slower neutrons are needed to speed up the reaction feels counterintuitive, don’t worry, I did as well when I first learned this. So feel free to just remember ‘moderators make the reaction more powerful’ and I will write the rest of this post in a way that doesn’t go further than that.) The most common moderator used today is water, but graphite has also historically been used.

For most reactors, each fuel rod is about 4.3 meters (14 feet) long, 200 to 250 fuel rods are bundled together into fuel assemblies, and 100 to 200 assemblies are assembled in a reactor’s core. This core sits inside a pressure vessel made of steel, concrete, and radiation-absorbing materials, and water is circulated through this vessel to take heat away from the fissioning core and generate electricity. This reactor vessel is located inside a containment building, an airtight structure of concrete, reinforced steel, and lead that provides one more layer of containment in the event of an emergency. Each fuel rod can provide energy for about six years and a single nuclear reactor can produce up to a gigawatt of power.
Once most of the nuclear fuel in a fuel assembly has been used up and a chain reaction can no longer be sustained, it is removed from the reactor. The uranium or plutonium fuel atoms have been broken apart into new isotopes that, while they can’t produce fission chain reactions, still produce a lot of heat and radiation through radioactive decay. When a fuel assembly has been freshly pulled out of the reactor, it will still be producing 7% of the energy it produced while fissioning, enough heat to melt the fuel rod and spread radioactive particles into the air. Now, the more radioactive a certain isotope is, the less time it will remain radioactive. After just a day, the fuel will be producing only one-twentieth as much energy as before. But the assembly will be hot enough to melt itself for years. So freshly spent fuel assemblies are immediately transferred to spent fuel pools, pools right next to the reactor that use constantly circulating water to keep the rods cool and shield their radiation. After five to ten years, the rods will be cool enough to be removed from the pools and be stored in concrete and metal casks. I’ll go into what happens next in a bit, but the long-lived parts of nuclear waste will remain radioactive enough to be dangerous for between ten thousand and a million years.
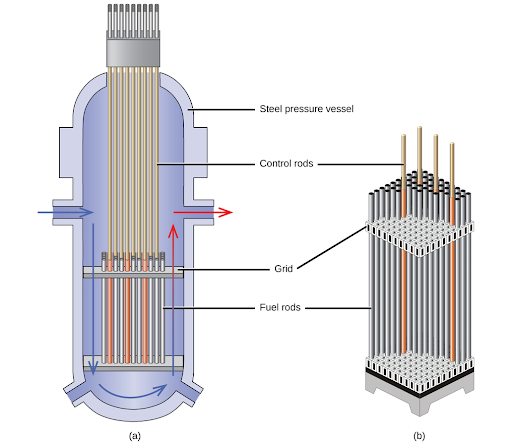
Risks and Benefits
For the past few decades, the usage of nuclear energy around the world has plateaued and in some regions declined. Germany and Japan have shuttered their nuclear programs over the past decade while the United States has only approved one new plant in the past 20 years. And while some of this decline can be attributed to replacement by renewable energy sources, the majority of this lost capacity has been picked up by coal and fossil fuels.
The main arguments against nuclear energy are well-known at this point. Japan shutting down most of its nuclear fleet was a direct response to the Fukushima-Daiichi disaster while the United States stopped building new plants shortly after the Three Mile Island disaster. I may talk next time about these disasters and what they can tell us about nuclear safety, but it’s worth putting these disasters in perspective. Of the 625 commercial power reactors that have ever been built, five* have failed in such a way as to release significant radiation into the surrounding environment. Statistically, nuclear energy causes 0.07 deaths for every terawatt-hour of electricity produced, most of these deaths being from radiation. To put that in perspective, 57.32 deaths occur for every terawatt-hour of electricity produced by coal, specifically from respiratory illnesses linked to breathing in pollution. This is followed by oil at 18.43 deaths per terawatt-hour and natural gas at 2.82 deaths per terawatt-hour. Another common argument against nuclear energy is what to do with the nuclear waste. Presently, the majority of this waste is piling up in temporary facilities and warehouses. There could be potential uses for this waste in the future, but the primary reason it’s being stored in this way is because there aren’t any places to permanently store it. Since this waste will be incredibly poisonous for up to a million years, a permanent solution will need to work without any human interference. (Slight tangent, but this video by Wendover Productions discusses the uniquely fascinating challenges that arise when planning around something that will be dangerous for longer than our species might exist.) But again, this isn’t unique to nuclear energy. Fossil fuels also produce waste that pose a significant long term threat to humanity. Unlike the waste products of fossil fuels, nuclear waste is solid with each fuel assembly weighing several tons, making it much easier to contain and store than carbon dioxide. And of course, there’s the threat that the technology used to make nuclear fuel can also be used to create nuclear weapons and that encouraging the use of nuclear energy could contribute to nuclear proliferation. This isn’t an unreasonable concern, but as we discussed last time, it is much harder to create weapons-grade uranium than reactor-grade uranium. Nuclear inspectors can easily tell if an enrichment plant is creating weapons-grade uranium just by looking at how the plant’s centrifuges are arranged. So long as treaties are enforced and followed, a nuclear energy plant being used to make weapons would at least be fairly conspicuous.
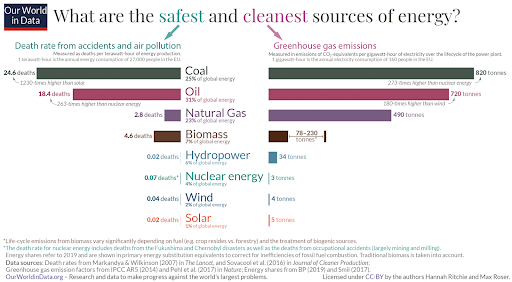
The drawbacks to nuclear energy that do carry some weight have less to do with safety and more with economics. The modern push for a renaissance of nuclear energy comes in part from environmentalists as an effort to combat climate change. To keep warming below 1.5°C above pre-industrial levels (the stretch goal of the Paris Climate Accord), global carbon emissions need to be cut in half by 2030. Nuclear energy is built on existing technology and produces no carbon dioxide. While even these proponents admit that our species’ long-term goal should be getting all of our energy from renewables (with current mining technology and energy demands, there’s only 250 years of minable uranium left on Earth), creating a 100% renewable energy grid will be difficult. Hydroelectric dams and geothermal plants can only be built in specific locations while wind and solar only work at specific times of day. High voltage transmission lines and pumped hydroelectric power storage can allow us to transport electricity long distance and ‘store’ electricity for future use, but building enough to make a completely renewable grid work would be a major infrastructure project on the scale of completely rebuilding our power grids. Nuclear plants could be a way to slash carbon emissions in the short-term to give ourselves more time to build a long-term solution.
But this isn’t a universal perspective and there are other environmentalists who question this logic. Nuclear power plants are by far the most expensive and time-consuming to build of any power producer. Constructing new nuclear plants require on average $6 to 9 billion USD per megawatt of capacity, more than twice that of a new coal plant. This makes nuclear energy less competitive against fossil fuels without government investment or carbon taxes. From groundbreaking to powering up, the smallest nuclear plants can take six to seven years to build, inspect, and certify, assuming everything goes to schedule. If the goal of nuclear energy is to reduce carbon emissions immediately, a solution that takes almost a decade for the first few plants to come online is a problem. So some have argued that the time and money that would be required to get these plants online would be better spent on the research and infrastructure to create better renewables.
It should be noted that the figures I cited above are global averages with a fair amount of variance. The fastest nuclear plant construction ever was the Kashiwazaki-Kariwa plant in Japan, built in only three years. The three fastest plant-building countries are Japan, South Korea, and China, with average build times of 46, 56, and 68 months respectively. China is the fastest growing producer of nuclear energy with 15 reactors currently under construction, followed by India with 8, and South Korea with 4. (Earlier when I said nuclear energy usage had plateaued, it’s more accurate to say some nations have rapidly built up their nuclear capacity while other nations have shuttered theirs.) While some have accused this of being due to less stringent regulations, which it might very well be the case for some countries, South Korea attributes their quick construction to modern construction techniques, standardized plant designs, and more involvement of safety inspectors during the planning and construction process. At the very least, it is possible to build nuclear reactors faster.
New Technologies
To end this on a positive note, I’ll talk about some technologies in development that could make nuclear energy safer, cheaper, or more productive. This is not to say these technologies will definitely become widely used or will fix every problem I’ve discussed above, but they at last have the potential to help significantly.
Small Modular Reactors
Small, self-contained reactors that produce only 300 megawatts. They’re small size means they could be mass produced in factories and transported by truck to power plants, significantly reducing construction times and cost. Some designs could also be safer than normal reactors as they would be sealed shut in the factory with fuel inside, leaving no space for radiation to escape. This video from Matt Ferrell goes into more detail.
Molten Salt Reactors
Commercial reactors today all use water to transfer heat from the fuel core to the steam source. The problem is that water boils at only 100°C, so if water ever stops being pumped through the core, it will start to boil off and the core will melt down. Pressurized water boils at much higher temperatures, but then the water escapes if there’s any small hole in the reactor. Most salts on the other hand have boiling temperatures of at least 1,400°C, meaning it’s much harder for a reactor to boil off its coolant even at normal pressure.
Thorium Reactors
Nuclear reactors aren’t only used for generating electricity. Breeder reactors are reactors designed to create nuclear fuel by bombarding certain elements with neutrons to change their isotope. Thorium reactors are designed to do both. There are multiple designs, but they all consist of a chamber of Uranium-233 inside a chamber of Thorium. About half the neutrons created by the U-233 bounce off the thorium, going back into the U-233 to trigger a nuclear chain reaction that generates heat and electricity. The other half of the neutrons are absorbed by the Thorium, turning it into more U-233. Uranium-233 is not found naturally anywhere on Earth, but it is very energy rich, is very difficult to weaponize, and its waste products stop being deadly after only a few centuries. Thorium is ten times as common in Earth’s crust as uranium, a lot of it being produced just from mining by-products. This video from Matt Ferrell goes into more detail about both thorium and molten salt reactors.
Deep Borehole Disposal
The current plan for nuclear waste is deep geological disposal; digging tunnels up to a kilometer deep, putting waste inside, and filling the tunnels back up. This is a very expensive process and can only be built in a select few locations, namely geologically stable locations with no ground water. While several such disposal sites have been planned or are being built, there is currently no such site in use anywhere on Earth. (The first will start receiving waste in 2023) This was what Yucca Mountain was meant to be, before the project was killed by budget constraints and nimbyism. So a proposal that has started gaining traction is to bury waste in boreholes similar to those used for oil drilling. Such a borehole could be dug far deeper than a traditional disposal site for far cheaper, and this depth means there are more safe places where these boreholes could be drilled. Experiments with this method have been promising and while there is still some skepticism among scientists and regulators (long-term waste disposal methods are necessarily held to high standards), this method has potential. This video from Deep Isolation (one company proposing this method) gives more details.
My goal with this post has been to give a more in-depth perspective on nuclear energy and the legitimate debate surrounding its usage. While I am personally in favor of expanding the use of nuclear energy, I acknowledge that this is an area where some reasonable debate can exist. At the very least, I feel comfortable saying that shutting down existing nuclear reactors is not a wise decision. While not perfect, nuclear energy is far greener than fossil fuels and is at present more reliable than most renewable energy sources. While renewables are the long-term future of energy, we will stand a much better chance of reaching that future if we keep all of our non-carbon producing options open. Climate change is a fight we need to win, so we shouldn’t fight with one hand tied behind our back.
For More Details
This entry is dedicated to Lindy Rhodes, who sadly passed away recently from feline lymphoma at age 16. She will be missed.
*I’m counting Fukushima-Daiichi three times for this figure as three of the plant’s six reactors released radiation. The other two instances were the Windscale fire and the Chernobyl disaster. I’m also not counting radiation releases that weren’t from power reactors, such as the Kyshtym disaster.
Comments