Nuclear Physics and Nuclear Energy, Part 1
- WSP Rhodes
- May 30, 2022
- 9 min read
With Chernobyl being in the news recently due to concerns surrounding radiation spikes following the invasion of Ukraine, it’s a good time to talk about nuclear science and energy. I’ve wanted to cover this topic for a while because of how much disinformation and ideology clouds this topic, particularly as it relates to the safety of nuclear energy. The reactor meltdown at Chernobyl and similar disasters have soured much of the public’s taste for nuclear energy and in research that would make nuclear energy safer. And while a healthy respect for such dangerous forces is certainly warranted, disasters like this are preventable with the right engineering and understanding of the science. My next post will go more into how these disasters happened, putting these disasters into perspective, and how nuclear energy should still play an important role in moving our society away from a dependence on fossil fuels. But this week, I will be going into the basic science of radiation and nuclear reactions, both for energy and for weapons, in order to make conceptualizing nuclear energy a bit easier.
What is Radiation?
I’ve touched on isotopes before (atoms with the same number of protons but different numbers of neutrons) and how they can be used in research. I’m sure you’ve all learned about the structure of the atom, but here is a short summary reminder. All atoms consist of a nucleus made up of protons and neutrons surrounded by a cloud of ‘orbiting’* electrons. The number of protons in an atom’s nucleus determines what element it is; e.g., an atom with six protons will always have the chemical properties of carbon. The positively-charged protons attract the same number of negatively-charged electrons and hold them in ‘orbit.’ The number of electrons ‘orbiting’ the nucleus determines what shape these ‘orbits’ take as they naturally position themselves as far apart as possible, and the shape of these ‘orbits’ determine how the atom interacts with other atoms, giving the atom its chemical properties. But because protons are positively-charged, they will repel each other powerfully if they get too close. To prevent an atom’s nucleus from ripping itself apart, non-charged neutrons sit between the protons so they don’t have to directly touch one another. The number of neutrons don’t affect the chemical properties of an atom (melting point, density, etc.), so it is possible for two atoms of the same element to have different numbers of neutrons with the only difference being a slight difference in mass.
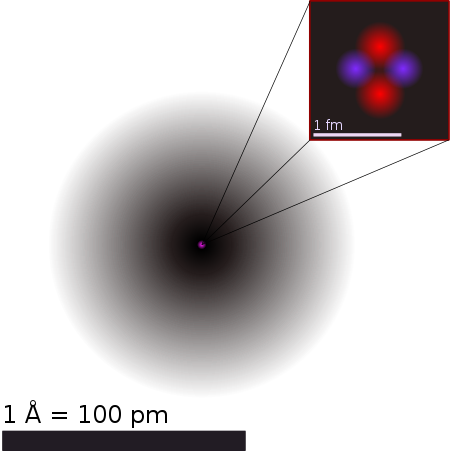
While there can be many different isotopes of any element, some isotopes are more stable than others. If an atomic nucleus has too few or too many neutrons, or is just too big, the nucleus won’t be able to permanently balance these magnetic forces. These unstable nuclei deal with this imbalance by tearing off the offending extra proton or neutron and pushing it away at near the speed of light, and it’s these fast moving particles torn off the nucleus that we call radiation. Because radiation particles are so fast, they have a lot of energy, so when they travel through complex molecules, such as our DNA, they can break these molecules apart.
Radioactive particles damage molecules by passing through an atom’s electron orbits, knocking away electrons and breaking apart molecular bonds. But this doesn’t necessarily mean it hits the atom’s nucleus; the electron ‘orbits’ are far larger than the nucleus, so atoms are mostly empty space. If an atom were the size of a football field, the nucleus would be the size of a quarter, so a radiation particle can move through hundreds of atoms and disrupt these ‘orbits’ without hitting any nuclei. But given enough time, many particles will hit another nucleus.** What happens next depends on the type of radioactive particle and how fast it’s moving, but under the right circumstances, the radioactive particle will become part of the new nucleus, changing the isotope and possibly the element of the atom. Sometimes, the new isotope is just as stable as the old isotope. Sometimes the isotope will also be an unstable, radioactive isotope and eventually decay and produce its own radiation. And sometimes, the new isotope will be so unstable that it immediately rips itself apart into smaller atoms, releasing a lot of energy in the process. This is called nuclear fission, and is the basis of nuclear energy and weaponry.
I would like to note that everything in the previous paragraph is an oversimplification. I’ve glossed over how radiation particles made from extra protons will have very different properties from those made from neutrons. There are also other types of radiation created when protons turn into neutrons and vice versa. And what a particular isotope will do when absorbing radiation depends on the type of radioactive particle and on the speed it hits with, which all depends on what decaying isotope it came from, and that can change the energy produced and the isotopes left behind. I will very briefly touch on neutron speed in my next post, but for now we’ll ignore it. This is a complicated field for a reason.
Nuclear Chain Reactions
The goal of any nuclear technology is to use radiation and radioactive isotopes to perform a task that is useful for humans. There are numerous applications for this in medicine and in scientific research, but I want to talk about nuclear energy, and by extension nuclear weapons. Both produce energy by creating a chain reaction within a mass of nuclear fuel where the radiation from decaying atoms set off more atoms decaying. For nuclear weapons, this reaction goes incredibly quickly, an entire mass of fuel decaying and releasing its energy in less than a second. For nuclear energy, this process goes much slower, the energy within the mass being released over the course of years.
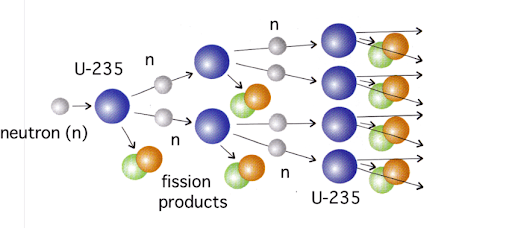
Setting this chain reaction up isn’t easy. First, one must identify isotopes that are fissile, meaning they can be set off by the radiation created by other atoms of said isotope. Uranium-235 (92 protons, 143 neutrons) is fissile because it will decay if it absorbs the radiation of other nearby U-235 atoms, turning into two smaller atoms and releasing neutrons as radiation. Uranium-238 won’t fission just from the radiation of other Uranium-238 atoms, so it doesn’t work as fission fuel.
Next, one must remove all the non-fissile atoms. While Uranium-235 atoms are the kind you want for making nuclear fuel, they account for less than 1% of all uranium atoms. Since the only difference between U-235 and U-238 is a slight difference in each atom’s mass and the atoms are all mixed evenly, separating the two is extremely difficult, which is why uranium enrichment is such a technical and expensive process. The most common process used today to enrich uranium involves taking smelted uranium metal, turning it into gaseous uranium hexafluoride via a series of chemical reactions, and spinning this gas in a centrifuge. Centrifugal force causes the heavier atoms to move toward the outer edge of the centrifuge and lighter atoms to move toward the center, so gas extracted from the center will contain more U-235. The difference in mass is incredibly slight, so each centrifuge has to spin at 1,500 revolutions per second to create the centrifugal force needed. And the gas that is removed from the center of each centrifuge has only 1% more U-235 than before, so this gas must be centrifuged multiple times to enrich the uranium enough to be useful. Depending on the reactor design, uranium to be used for nuclear energy needs to be between 3% and 20% U-235, while uranium for nuclear weapons needs to be as close to pure U-235 as possible. This means that it is impossible to turn a nuclear reactor into a nuclear bomb or to use the fuel from a nuclear reactor to make a nuclear bomb. The goal of a nuclear reactor is to set up a scenario where each decaying atom sets off one other decaying atom, so the energy is released bit by bit. For a nuclear bomb, the goal is to set up a scenario where each decaying atom sets off at least two other decaying atoms. This means that each ‘generation’ is bigger than the last; one atom decaying sets off two atoms which set off four atoms, etc., so the energy locked up in the fuel is completely released in less than a second.
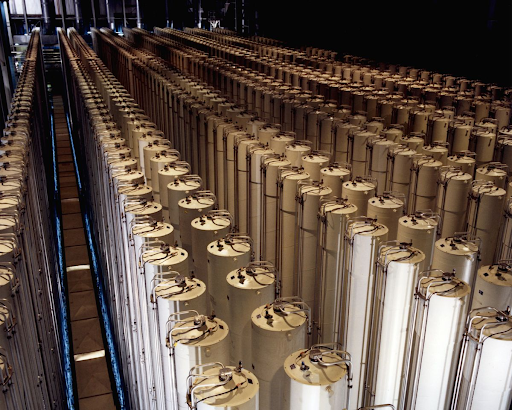
Once enough fissile material has been enriched and repurified into solid metal, the final stage is to create a nuclear chain reaction. The goal is to create a space where the radiation from each decaying atom triggers another atom to decay, like a chain of dominoes. But as I mentioned before, atoms are mostly empty space so most radiation that is created within a chunk of enriched uranium will escape the chunk without hitting another atomic nucleus and setting off another reaction. Even if a stray neutron does set off another atom decaying, the odds are very low that any of this new radiation will set off another round of decay. To ensure each decay sets off another, a critical mass of nuclear fuel must be created. This is the amount of radioisotope needed in one place to guarantee each stray neutron will run into another nucleus before it can escape the mass. The critical mass for Uranium-235 is 52 kilograms*** (about 115 pounds), so this is how big a chunk of enriched uranium one needs to guarantee a sustained chain reaction will occur. The Hiroshima bomb consisted of two chunks of uranium, each slightly below critical mass, propelled into each other with chemical explosives. When they collided, a critical mass was created, causing a nuclear chain reaction that released 63 terajoules of energy in less than a second. This energy, released primarily as a burst of x-rays, heated the surrounding air to 7,700°C in less than a second, hotter than the surface of the sun. The flash alone was hot enough to ignite any flammable substance, including human skin, within two kilometers (1.24 miles) of the blast. Not-so-fun fact; most of the enriched uranium fuel in the Hiroshima bomb didn’t actually detonate as it was vaporized and blown away from the explosion before it could explode itself. The amount of fuel that successfully fissioned, that destroyed two-thirds of a major city and killed 80,000 people, weighed about 0.7 grams, less than a banknote.
Effects of Radiation
Let’s conclude by going back to radiation itself. Radiation particles can damage whatever complex molecules they pass through, but DNA is particularly susceptible. Enough radiation can overtax the complex mechanisms that cells use to repair damage to their DNA. A large enough dose of ionizing radiation can create more DNA damage than cells can repair, resulting in mutations. As I’ve discussed before, certain mutations can make other mutations more probable, eventually leading to cells becoming cancerous. The larger the dose of radiation, the more mutations can occur and in more cells, leading to a higher risk of cancer with less time before it manifests. And if the dose of radiation is very high, it can lead to radiation poisoning. Radiation damages enough DNA that some cells die, particularly fast-growing cells that don’t have time to repair their DNA before they reproduce. The number of blood cells in the body drop precipitously, leading to anemia and opportunistic infections caused by a weakened immune system. The lining of the digestive tract becomes damaged, causing nausea, vomiting, and diarrhea. Skin damage can occur, particularly skin that came into contact with the radiation source, particularly blisters, ulcers, and peeling. Radiation poisoning can be treatable if the dose is low enough, though increased cancer risks would still occur, but large enough doses have a 100% fatality rate.
The good news is that, just like with anything toxic, the danger posed by radiation is all about dosage. Between radiation from space and from sources on Earth, the average human receives about 10 microsieverts of ionizing radiation per day. The slight amount of DNA damage caused by this much radiation is easily repaired. A chest X-ray will give one between 20 and 100 microsieverts at once; sudden acute radiation exposure is more dangerous than gradual exposure, but 100 microsieverts is still a very small dose. A transatlantic flight will expose you to about 40 microsieverts as there is less atmosphere above you to filter out cosmic radiation. This does give career pilots and airplane crews a significantly higher dosage than most people, receiving up to 9,000 microsieverts a year as opposed to the average of 4,000 microsieverts per year. This dosage is considered when determining schedules for airline workers, but it is still well below the 50,000 microsieverts the EPA sets as a maximum radiation dosage a worker can be exposed to in a year. 100,000 microsieverts a year is the lowest dosage that has been definitively linked with an increased lifetime cancer risk and 400,000 microsieverts in a short period of time is the threshold where radiation poisoning starts to set in. This xkcd comic provides a good visual aid of radiation dosage. All of this is to say that radiation is omnipresent in our universe and while it does present an inherent danger that should be respected, it’s a danger that is understood and can be managed and mitigated.
Hopefully, this has all provided some context for how nuclear energy works on a scientific level. My next post will use what we’ve talked about here to discuss how nuclear reactors are designed and built, what failures like Chernobyl tell us about how they can be designed better, and how these failures don’t mean the technology is too dangerous to use.
For More Details
*I keep putting electron ‘orbit’ in parentheses because these are not orbits like you see with planets. The exact form electron orbits involve more quantum mechanics than either of us would like to talk about here. But for now, picture electron orbits as a ‘cloud’ surrounding the nucleus, made up of electromagnetic charges created by moving electrons.
** It’s exclusively neutron radiation, radiation consisting of single neutrons, that can be absorbed by other nuclei. This is because neutrons don’t have any electromagnetic charge, so they can’t be magnetically repelled or deflected by the charges of the atom.
*** This critical mass can be lowered by increasing the density of the fuel or surrounding it with neutron reflectors. The Nagasaki bomb and modern nuclear weapons work by crushing a sphere of nuclear fuel with powerful explosives, momentarilly packing the fuel’s atoms together so tightly that radiation can’t help but trigger a chain reaction.
Comments